Featured Experts
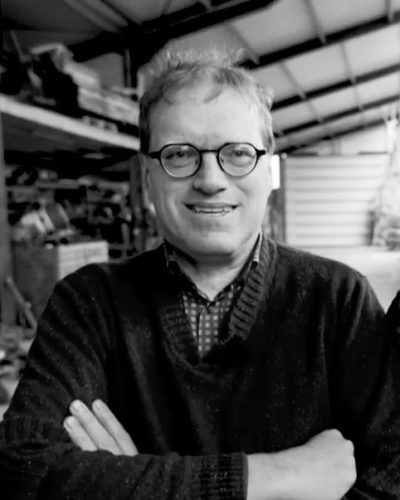
Pol Knops
Founder of Green Minerals
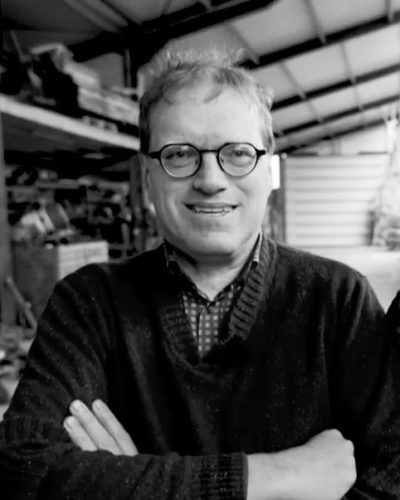
Pol Knops
Founder of Green Minerals
Pol Knops is the Chief Technology Officer and Founder of Green Minerals, a tech company using olivine to mineralize CO2 and turn it into functional products like concrete and paper.
In this Episode
There are several methods of removing carbon dioxide from the atmosphere – both natural and technological – and each method has its tradeoffs.
Carbon mineralization is the most secure option for carbon dioxide removal (CDR) as it permanently sequesters CO2 in the crystal structure of minerals.
But the natural mineralization process is too slow to keep pace with the rate of manmade CO2 emissions. So scientists are finding ways to speed up the process.
In Climate Now’s latest episode of our series on carbon dioxide removal strategies, we explore how the mineralization process works and how it can be enhanced to remove carbon dioxide from the atmosphere on a large scale to help mitigate climate change.
Related Media:
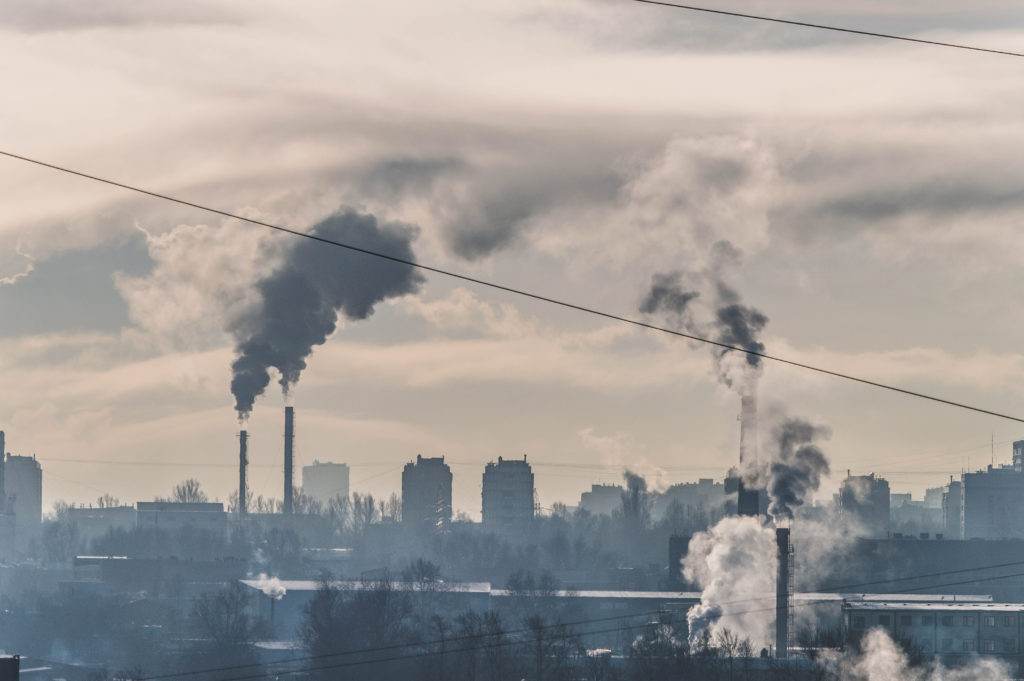
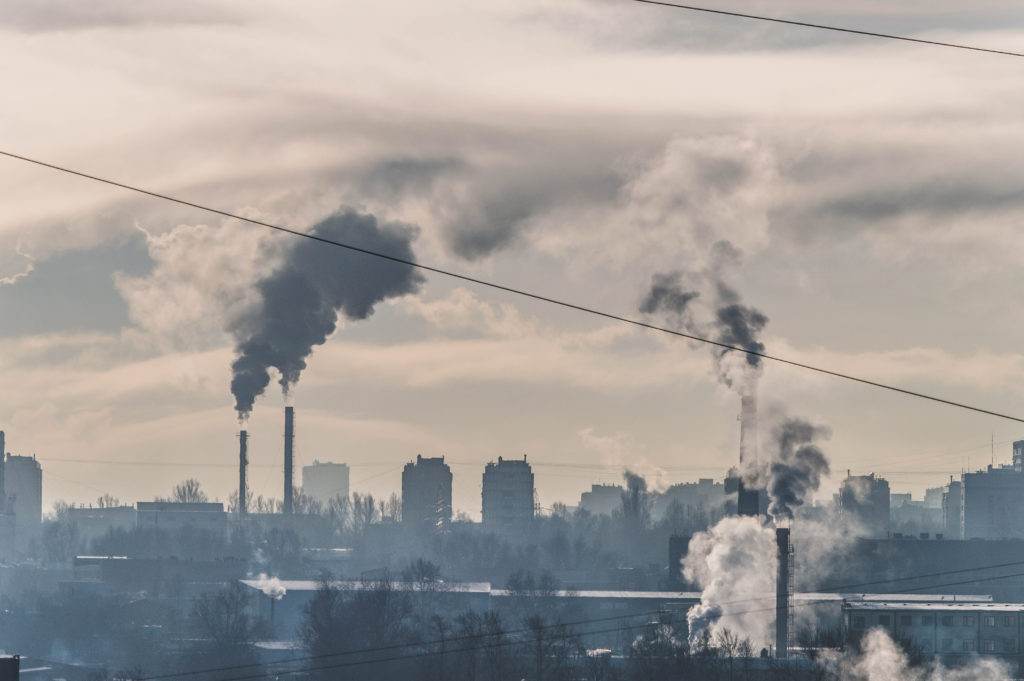
A Climate Change Primer Ep 7
Carbon Dioxide Removal (CDR)
In order to reach global net-zero emissions by 2050, we must remove CO2 from the atmosphere as well as prevent further emissions. Carbon Dioxide Removal (CDR) can be accomplished naturally – through forests, soil sequestration, or mineralization –
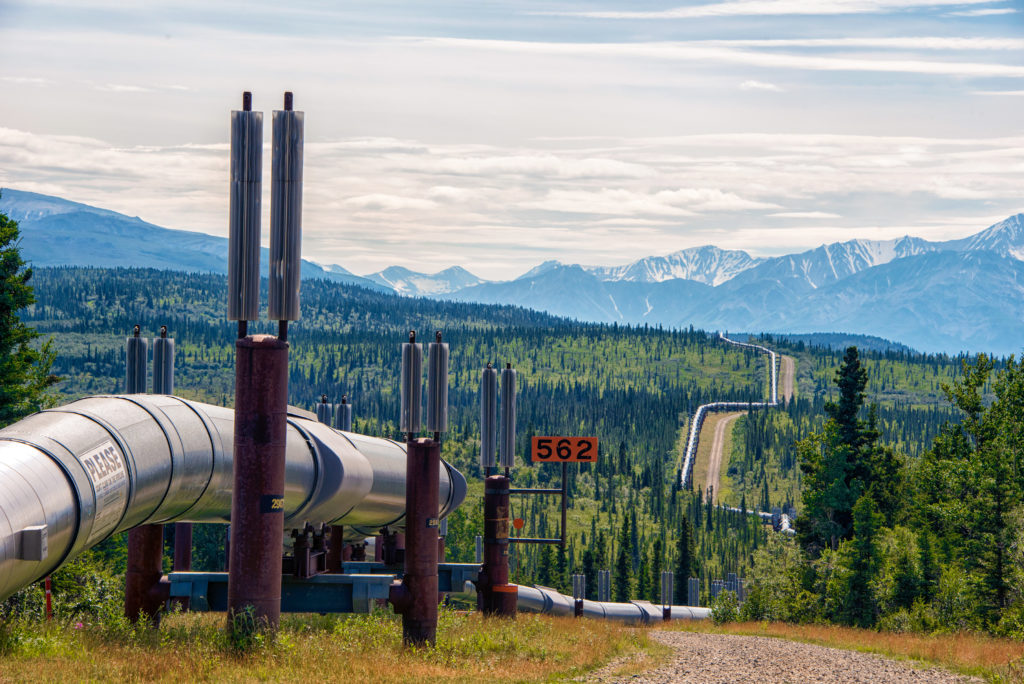
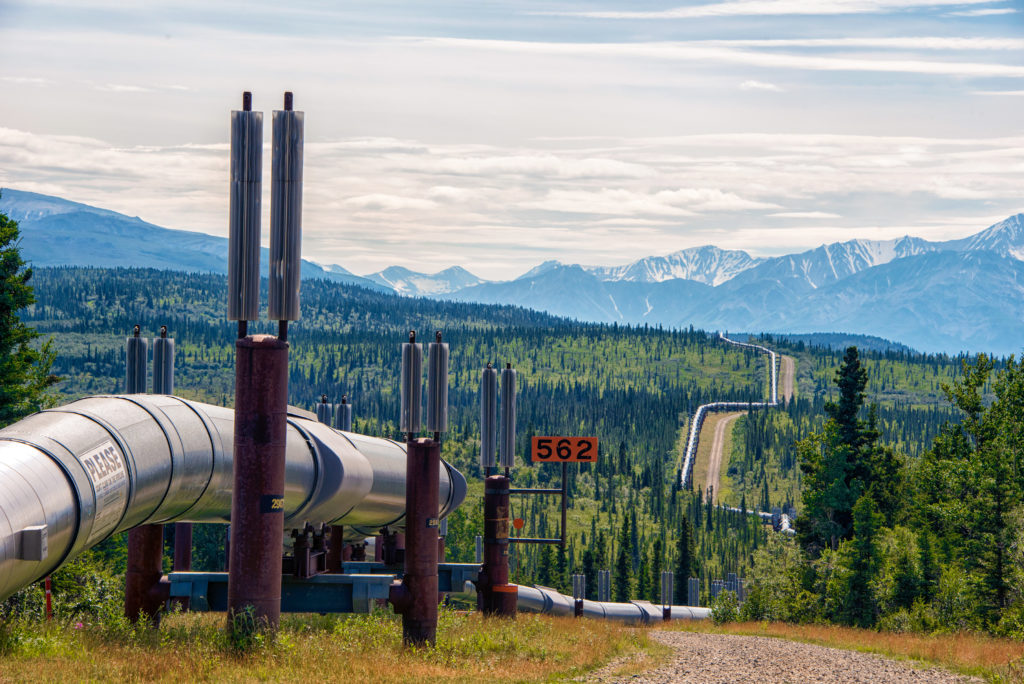
Technologies Ep 6
Carbon Dioxide Removal: Carbon Capture and Storage, Part II
Carbon Capture and Storage (CCS) has the potential to remove billions of tons of CO2 from the atmosphere annually, which we will likely need to reach global climate targets. In the first of our two-part series on CCS, we explored how carbon capture technol
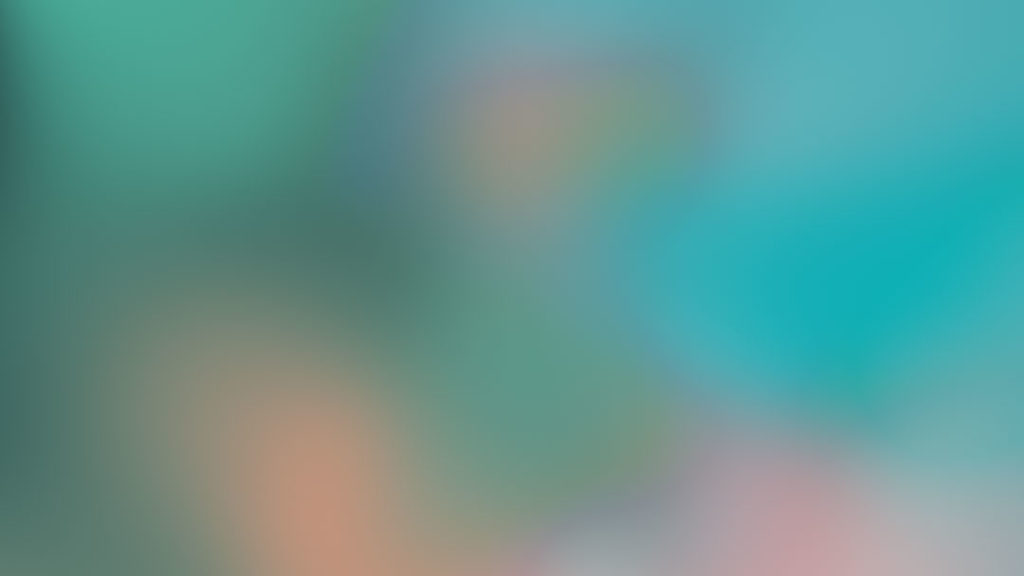
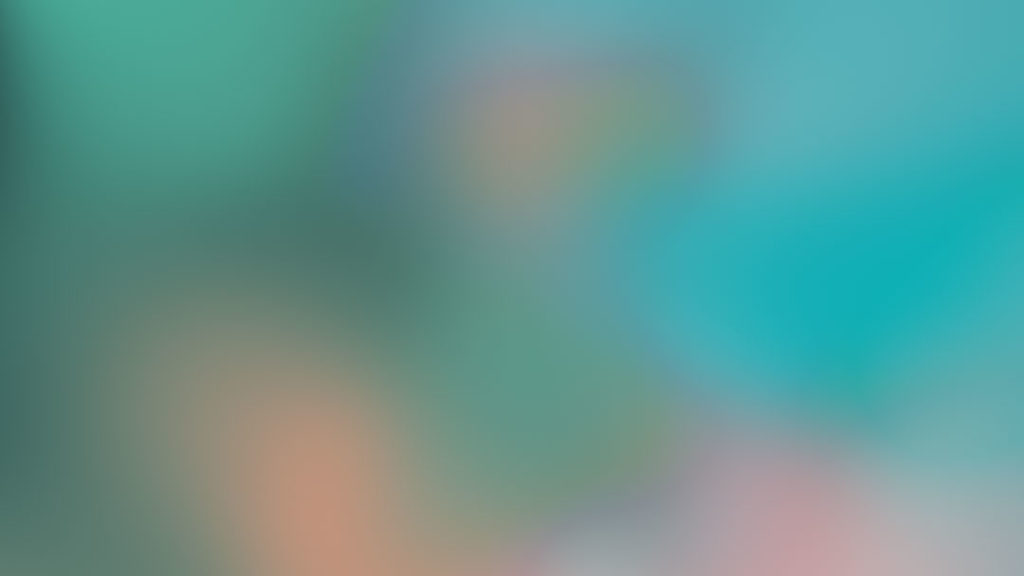
Climate Now: Oct 25, 2021
Trash to treasure: One man’s journey to make CO2 waste a useful product
What does it take to turn an idea that could help fight climate change into a self-sustaining business? We often hear the glamorous stories of startups that have made it, but little about the struggles, the learning, and the luck required to get there. Pol Kno
Episode Transcript
10 billion tonnes a year. That is how much carbon dioxide we will likely need to remove from the atmosphere every year by 2050 to avoid catastrophic changes to our climate. By 2100, we will need to remove 20 billion tons per year. And we have some choices in how we remove this CO2, as we describe in our introductory video on Carbon Dioxide Removal. But the technique we choose will invariably require a trade-off:
We can choose the most cost-efficient options, or we can choose those methods that will come with the strongest guarantee of long-term CO2 storage. In this video, we focus on the most secure of CDR options: Mineralization.
Mineralization is like the holy grail of CDR technologies because it traps CO2 into the crystal structure of minerals, thereby permanently sequestering it from the atmosphere. Every other proposed reservoir for CO2 storage comes with some risk of impermanence:
- Forests can burn or be destroyed by pests.
- Soils that are cultivated to hold more CO2 will lose it again if land users revert to earlier, more destructive practices.
- Even storage of CO2 in the pore spaces of underground geologic formations could escape through abandoned wells or along fractures in the rocks.
But with mineralization, CO2 becomes a part of a rock. There is nearly zero risk of it being re-released to the atmosphere. So let’s dig deeper into the technology – how does it work? And how feasible is it to develop this method as a major pillar of carbon dioxide removal?
Removing CO2 from the atmosphere through mineralization is NOT a novel technique. In fact, it is as old as the Earth itself. Mineralization is the primary mechanism by which the planet regulates atmospheric CO2 over timescales of millions of years.
Whenever it rains, CO2 in the atmosphere reacts with water in raindrops, forming a compound called carbonic acid.
As the rain lands on Earth’s surface, the carbonic acid reacts with exposed rocks, slowly dissolving the minerals by breaking them down into their metal components. Those dissolved components then get carried by runoff and river water, and are ultimately released into the ocean. And because seawater has a slightly basic pH, when these dissolved metals enter the ocean, they react with carbon dioxide in the ocean to form a carbonate mineral, trapping the CO2 into the mineral structure.
This process can happen inorganically, but in oceans today, most carbonate precipitation is facilitated by organisms, who use calcium carbonate to create their shells or skeletons. Every clam, coral and microscopic foraminifera on Earth is doing its part to sequester CO2 into its mineralized hard parts. And when these organisms die, and their shells and skeletons settle on the seafloor and get buried, they are taken out of contact with Earth’s atmosphere entirely. It will take tens or hundreds of millions of years of plate tectonic processes like mountain building for those carbonate minerals to reach the surface again, where they could be exposed to rain and dissolve.
In fact, the formation and burial of carbonate minerals is so effective, that there is more than 10,000 times as much carbon stored as minerals in sedimentary rocks as there is carbon in the form of CO2 in the atmosphere.
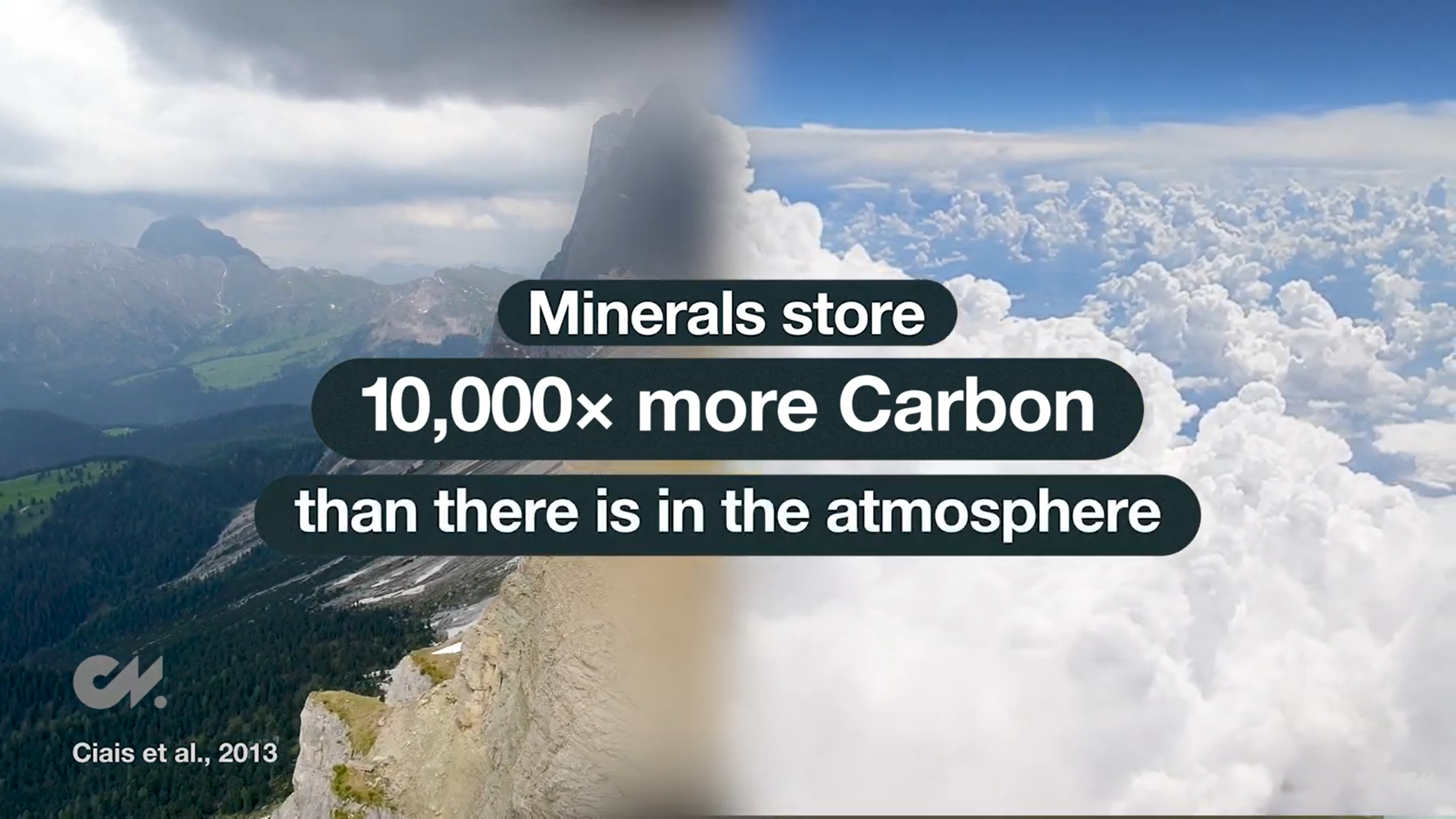
Unfortunately, mineralization within the Earth’s natural carbon cycle occurs on very slow timescales, that cannot keep pace with the rates of man-made emissions. At most, natural mineralization removes about 0.7 billion tonnes of carbon dioxide from the atmosphere every year. We emit nearly 40 billion tonnes of carbon dioxide each year. But, what if we could find a way to simulate this natural process, and speed it up?
Pol Knops is a global leader in the effort to engineer a climate change solution that mirrors the natural carbonate mineralization process. Coming from a background in physics, renewable energy and waste systems, he realized that you could build a high-pressure, high-temperature reactor that mimics the natural mineralization process.
By reacting CO2 and water with powdered rock in Mr. Knops’ reactor, he is able to recreate the natural processes of rock dissolution and carbonate mineral precipitation that happen in the natural CO2 cycle. At higher pressures and temperatures, the reaction proceeds more rapidly. And by powdering the rock, he is able to maximize the reactive surface area of the starting material, which also accelerates the reaction.
Another advantage of engineering carbonate mineral reactions is that we can choose our starting material. Rocks that are rich in the minerals olivine, pyroxene and plagioclase, like the volcanic basalts found in Hawaii and Iceland, are far more reactive than average continental rocks. This is because these minerals are rich in calcium, magnesium and iron, the metals that react with CO2 to form carbonate minerals. And luckily, we have an abundance of basaltic rock on our planet.
The upper few kilometers of ocean crust – which covers 70% of Earth’s surface – is made almost entirely out of basalt, so anywhere that the ocean crust hasn’t been appreciably buried by sediment is a potentially viable mineralization site. But even considering only on-land rock reservoirs, the potential capacity for carbonate mineralization is orders of magnitude more than we need.
To remain below 1.5oC of warming, we will need to remove a total of about 125 billion tonnes of CO2 out of the atmosphere by the end of this century. For comparison, there is about 12 thousand billion tonnes of CO2 mineralization potential using rocks in the United States and Europe alone, enough to store a century’s worth of anthropogenic emissions. Globally, the rock reservoir capacity for mineralization is 60 BILLION billion tonnes of CO2, so the material is there. We just have to figure out the most energy efficient and cost effective way to use it.
Generally, proposed mineralization techniques fall into two categories, which we will explore separately.
- EX SITU mineralization involves extracting rock from the ground to react with CO2, whereas
- IN SITU mineralization involves pumping CO2 underground to react with subsurface rocks.
Let’s take a look at how ex situ mineralization works, first. This technique can be one of the most expensive carbon dioxide removal strategies, costing as much as 600 US dollars per tonne of CO2. This is because to remove a high volume of CO2 at a fast rate requires lots of fresh rock, and lots of energy to power the mineralization reaction.
There are a few ways to reduce these costs, but each approach decreases the realistic capacity of this type of sequestration. Let’s consider the reactive material first. For every ton of CO2 we capture, we need at least 1.6 tonnes of fresh magnesium- and calcium-rich rock. We could mine this rock from the ground and mechanically crush it, which is the most expensive and energy intensive option.
Or, we could use existing rock waste, either from industrial processes or from the tailings of old mining operations. This could bring the price of mineralization down to $50 per tonne. But, there is only so much waste material available. The MOST optimistic estimates would provide about 1.3 billion tons of CO2 storage annually, only about 10% of what is needed.
Another approach is to develop a source of revenue to offset the cost of energy and primary materials. This is what Mr. Knops’ company, Green Minerals, does, by selling the carbonate and silica byproducts of the mineralization reaction.
Pol Knops:
“If you make paper in Europe there’s about 6 million tons of lime for paper manufacturing. They add lime for a few reasons, first of all for the goal of making it more white, but more importantly to make it cheaper and have better properties, and the idea is, instead of using the traditional lime, to use this material.“
Finding a market like paper, which values mineralization byproducts at prices over $100 per tonne, could make mineralization a financially viable industry. But, how big could this industry be?
The European paper market for lime can sequester about 2 million tons of CO2 annually if replaced entirely with mineralization byproduct. That market would need to scale up 5,000 times to reach the 10 gigatons of annual CO2 storage we need by 2050.
Even if mineralization completely replaced the global market for lime, which in 2020 was 420 million metric tons, it would sequester less than 2% of the CO2 needing to be removed annually. There is simply not a large enough market to finance CO2 reduction at the required scale.
One final approach to reducing the cost of ex situ mineralization is to forego using an energy-intensive reactor. Instead, finely ground powders of reactive rock is spread on beaches, croplands or surface repositories. This approach, called enhanced weathering, increases the surface area of reactive rock in contact with rain, speeding up the natural CO2 weathering cycle. Enhanced weathering can cost less than 10 US dollars per tonne of CO2, if mining waste is used, or 50 to 200 dollars per tonne if freshly mined rock is used. And, this technique could be employed at a large scale. For example, if we covered all 166 million hectares of agricultural land in the US with a 1 centimeter layer of powdered olivine, it could sequester about 3.4 billion tonnes of CO2. However, a lot of uncertainty remains with how this technique would work in reality. First, it is not clear how quickly the powdered material would actually break down. And, other, possibly hazardous, metals could also leach from the powdered rock into the soil during weathering.
So, let’s consider our other option: the potential for in situ mineralization.
The good news about this technique is that the subsurface rocks don’t need to be mined, and are already under temperature and pressure conditions ideal for mineralization. That means that costs can be much lower than ex situ techniques, ranging from about 10 to 30 US dollars for on-land storage sites. And, the potential storage capacity is orders of magnitude more than we need.
However, testing how well in situ mineralization actually works is still in its nascent stages. CarbFix, a collaborative research and industry project in Iceland, built the world’s first carbon capture plant utilizing mineralization in 2014. The plant works in tandem with an existing hydrothermal power plant a top Iceland’s basalt-rich crust. It can scrub CO2 from gases released from the geothermal energy plant or the ambient air. The CO2 is mixed with hot water being pumped for electricity production at the powerplant, and the mixture is then pumped into the hot basalt subsurface. There, the CO2-water mix travels through pore spaces and fractures in the rock, reacting to form carbonate minerals.
At the Carbfix site, over 95% of the injected CO2 mineralized in less than 2 years. The six year pilot program, which is now sequestering carbon at a rate of about 10,000 metric tons per year, has optimized the process such that costs are around 25 U.S. dollars per ton of CO2.
This low cost is due in part to the ideal location of the CarbFix project, and costs may be higher in regions less rich in water and geothermal energy resources. But, CarbFix provided the proof of concept that in situ mineralization can be affordably achieved.
In sum, the possibilities of CO2 mineralization are as promising as the approaches are diverse. However, like other methods of carbon capture and storage, mineralization will require significant upfront investment and government support.
It must also be noted that the range in price that we examined for mineralization, from 10 to 600 US dollars per tonne, only represents the STORAGE costs of CO2. Most of these techniques require that carbon dioxide is first captured from an emission source or from ambient air, and then transported to the mineralization site. Those capture and transport costs can range from 25 dollars to thousands of dollars per ton, as we detail in our related videos on Carbon Capture and Sequestration.
To learn more, you can find our other carbon capture and sequestration videos at our website, ClimateNow.com. And, listen to the complete story of how Green Minerals is helping build a mineralization industry, in our full podcast with Pol Knops.
Thanks and see you next time!
SOURCES
- 00:14, 6:14 National Academies of Sciences, Engineering, and Medicine (2019) Negative Emissions Technologies and Reliable Sequestration: A Research Agenda. Washington, DC: The National Academies Press. https://doi.org/10.17226/25259
- 1:06 Matter, J.M., et al. (2016) Rapid carbon mineralization for permanent disposal of anthropogenic carbon dioxide emissions. Science, 352, 1312-1314. https://doi.org/10.1126/science.aad8132
- 1:14 Fuss, S., et al. (2018) Negative emissions–Part 2: Costs, potentials and side effects. Environmental Research Letters, 13, 063002. https://doi.org/10.1088/1748-9326/aabf9f
- 2:04 Walker, J.C.G., P.B. Hays, J.F. Kasting (1981) A negative feedback mechanism for the long-term stabilization of Earth’s surface temperature. Journal of Geophysical Research, 86, 9776-9782. https://doi.org/10.1029/JC086iC10p09776
- 2:13 Kasting, J.F. and J.C. Walker (1992) The geochemical carbon cycle and the uptake of fossil fuel CO2. AIP Conference Proceedings, 247(1), 175-200. American Institute of Physics. https://doi.org/10.1063/1.41927
- 2:13 Dunsmore, H.E. (1992) A geological perspective on global warming and the possibility of carbon dioxide removal as calcium carbonate mineral. Energy Conversion and Management, 33(5-8), 565-572. https://doi.org/10.1016/0196-8904(92)90057-4
- 2:20, 2:52 Krauskopf, K.B., & D.K. Bird (2003) Introduction to Geochemistry, 3rd Ed. New York: McGraw Hill Custom Publishing.
- 3:05 Reference Terms: Limestone. Science Daily. Published online (no date). Accessed November 8, 2021. https://www.sciencedaily.com/terms/limestone.htm
- 3:48, 4:09 Ciais P., et al. (2013) Carbon and other biogeochemical cycles. In Stocker, T.F., et al. (Eds.), Climate Change 2013: The Physical Science Basis. Contribution of Working Group I to the Fifth Assessment Report of the Intergovernmental Panel on Climate Change (pp. 465-570). Cambridge, UK, and New York, NY, USA: Cambridge University Press. https://www.ipcc.ch/report/ar5/wg1/
- 4:11 Ritchie, H. and M. Roser. CO2 emissions. Our World in Data. Published online (no date). Accessed November 8, 2021. https://ourworldindata.org/co2-emissions
- 5:10, 6:38, 7:04, 7:18, 8:08, 8:20, 10:20, 11:11, 11:35, 13:19 Kelemen, P., et al. (2019) An overview of the status and challenges of CO2 storage in minerals and geological formations. Frontiers in Climate, 1, 9. https://doi.org/10.3389/fclim.2019.00009
- 4:25 video courtesy Pol Knops
- 5:17 Olivine, Rob Lavinsky, iRocks.com – CC-BY-SA-3.0
- 5:18 Pyroxene, Rob Lavinsky, iRocks.com – CC-BY-SA-3.0
- 5:20 Plagioclase, licensed under public domain
- 5:45 Snæbjörnsdóttir, S.Ó., et al. (2020) Carbon dioxide storage through mineral carbonation. Nature Reviews, 1, 90-102. https://doi.org/10.1038/s43017-019-0011-8
- 6:25 Where does it work? Carbfix.com. Published online (no date). Accessed November 11, 2021. https://www.carbfix.com/atlas
- 7:48, 11:11 The most efficient reaction is 2CO2 + Mg2SiO4(olivine) → 2MgCO3(carbonate) + SiO2(quartz). This reaction requires 2 mols CO2 (88g CO2) and 1 mol olivine (144g olivine). Normalized to 1kg CO2, the mineralization reaction requires 1.6 kg olivine. Basalt, which is significantly poorer in (Ca+Mg+Fe), could require as much as 3 times as much starting material to sequester the same amount of CO2 Renforth, P. (2012) The potential of enhanced weathering in the UK. International Journal of Greenhouse Gas Control, 10, 229-243. http://dx.doi.org/10.1016/j.ijggc.2012.06.011 Fig. 1, p. 230
- 9:10 personal communication, Pol Knops
- 9:40 U.S. Geological Survey, Mineral Commodity Summaries, Lime. Prepared by Apodaca, L.E., January 2021. https://pubs.usgs.gov/periodicals/mcs2021/mcs2021-lime.pdf
- 10:08, 10:44, 11:11 Beerling, D.J., et al. (2020) Potential for large-scale CO2 removal via enhanced rock weathering with cropland. Nature, 583, 242-248. https://doi.org/10.1038/s41586-020-2448-9
- 10:37 Map of croplands in the United States. USGS. Published online (no date). Accessed November 11, 2021. https://www.usgs.gov/media/images/map-croplands-united-states
- 11:54, 12:38 We turn CO2 into stone. Carbfix. Published online (no date). Accessed November 11, 2021. https://www.carbfix.com/
- 12:12 Gutknecht et al. (2018, Energy Procedia) https://doi.org/10.1016/j.egypro.2018.07.017